Excitonium
After 50 years of theories and thwarted attempts, scientists have finally proved the existence of a new form of matter. The never-before-detected condensate is called excitonium, a name first coined in the 1960s by Harvard theoretical physicist Bert Halperin, who is now 76. Peter Abbamonte is the physicist responsible for the Discovery.
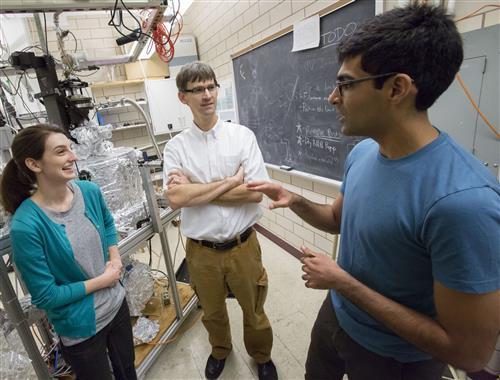
Abbamonte and graduate students Anshul Kogar and Mindy Rak, with input from colleagues at Illinois, University of California, Berkeley, and University of Amsterdam group achieves first-ever measurement of excitonium collective modes and first observation of soft plasmon in any material.
The team studied non-doped crystals of the oft-analyzed transition metal dichalcogenide titanium diselenide (1T-TiSe2) and reproduced their surprising results five times on different cleaved crystals. University of Amsterdam Professor of Physics Jasper van Wezel provided crucial theoretical interpretation of the experimental results.
Excitonium is a condensate — it exhibits macroscopic quantum phenomena, like a superconductor, or superfluid, or insulating electronic crystal. It’s made up of excitons, particles that are formed in a very strange quantum mechanical pairing, namely that of an escaped electron and the hole it left behind.
It defies reason, but it turns out that when an electron, seated at the edge of a crowded-with-electrons valence band in a semiconductor, gets excited and jumps over the energy gap to the otherwise empty conduction band, it leaves behind a “hole” in the valence band. That hole behaves as though it were a particle with positive charge, and it attracts the escaped electron. When the escaped electron with its negative charge, pairs up with the hole, the two remarkably form a composite particle, a boson—an exciton.
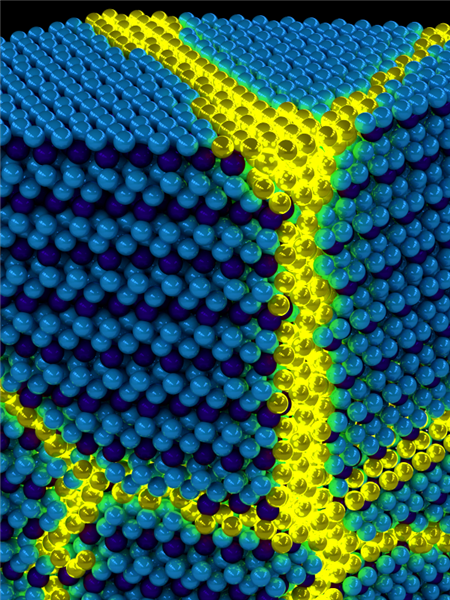
In point of fact, the hole’s particle-like attributes are attributable to the collective behavior of the surrounding crowd of electrons. But that understanding makes the pairing no less strange and wonderful.
Until now, scientists have not had the experimental tools to positively distinguish whether what looked like excitonium wasn’t in fact a Peierls phase. Though it’s completely unrelated to exciton formation, Peierls phases and exciton condensation share the same symmetry and similar observables—a superlattice and the opening of a single-particle energy gap.
Abbamonte and his team were able to overcome that challenge by using a novel technique they developed called momentum-resolved electron energy-loss spectroscopy (M-EELS). M-EELS is more sensitive to valence band excitations than inelastic x-ray or neutron scattering techniques. Kogar retrofit an EEL spectrometer, which on its own could measure only the trajectory of an electron, giving how much energy and momentum it lost, with a goniometer, which allows the team to measure very precisely an electron’s momentum in real space.
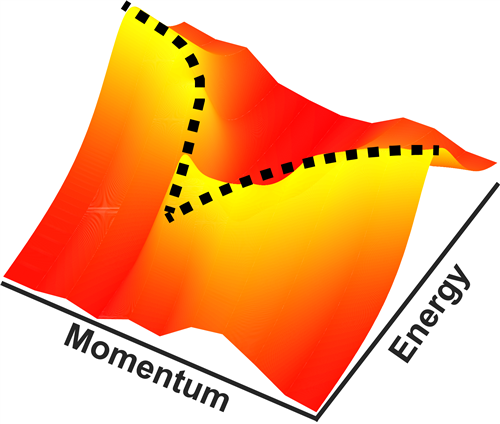
With their new technique, the group was able for the first time to measure collective excitations of the low-energy bosonic particles, the paired electrons and holes, regardless of their momentum. More specifically, the team achieved the first-ever observation in any material of the precursor to exciton condensation, a soft plasmon phase that emerged as the material approached its critical temperature of 190 Kelvin. This soft plasmon phase is “smoking gun” proof of exciton condensation in a three-dimensional solid and the first-ever definitive evidence for the discovery of excitonium.
“This result is of cosmic significance,” affirms Abbamonte. “Ever since the term ‘excitonium’ was coined in the 1960s by Halperin and Rice, physicists have sought to demonstrate its existence. Theorists have debated whether it would be an insulator, a perfect conductor, or a superfluid—with some convincing arguments on all sides. Since the 1970s, many experimentalists have published evidence of the existence of excitonium, but their findings weren’t definitive proof and could equally have been explained by a conventional structural phase transition.”
Rak recalls the moment, working in the Abbamonte laboratory, when she first understood the magnitude of these findings: “I remember Anshul being very excited about the results of our first measurements on TiSe2. We were standing at a whiteboard in the lab as he explained to me that we had just measured something that no one had seen before: a soft plasmon.”
“The excitement generated by this discovery remained with us throughout the entire project,” she continues. “The work we did on TiSe2 allowed me to see the unique promise our M-EELS technique holds for advancing our knowledge of the physical properties of materials and has motivated my continued research on TiSe2.”
Kogar admits, discovering excitonium was not the original motivation for the research — the team had set out to test their new M-EELS method on a crystal that was readily available — grown at Illinois by former graduate student Young Il Joe, now of NIST. But he emphasizes, not coincidentally, excitonium was a major interest:
“This discovery was serendipitous. But Peter and I had had a conversation about 5 or 6 years ago addressing exactly this topic of the soft electronic mode, though in a different context, the Wigner crystal instability. So although we didn't immediately get at why it was occurring in TiSe2, we did know that it was an important result—and one that had been brewing in our minds for a few years."
The team’s findings was published in the December 8, 2017 issue of the journal Science in the article, “Signatures of exciton condensation in a transition metal dichalcogenide.”
This fundamental research holds great promise for unlocking further quantum mechanical mysteries: after all, the study of macroscopic quantum phenomena is what has shaped our understanding of quantum mechanics. It could also shed light on the metal-insulator transition in band solids, in which exciton condensation is believed to play a part. Beyond that, possible technological applications of excitonium are purely speculative.
Spontaneous Bose-Einstein condensation of excitons
Excitons are pairs of electrons and holes inside a solid material that together behave like a single particle. It has long been suspected that when many such excitons exist in the same piece of matter, they can form a single giant quantum state called a Bose-Einstein condensate – the same process which is responsible for a metal losing all its electrical resistance when it becomes a superconductor, for example.
In the early 20th century, physicists discovered that the world around us consists of two types of particles: bosons and fermions. The main difference between these particles is how they behave when one tries to bring them into the same physical state, with the same position, the same velocity, and so on. While for two fermions (such as electrons) it is fundamentally impossible to ever be in the exact same state, two or more bosons (such as photons, particles of light) can be in the same state at the same time without any problems. In fact, at low enough temperatures, collections of bosons will prefer such a situation: the particles have the tendency to all occupy the same state, in a process known as Bose-Einstein condensation.
Excitons
For most types of bosons, Bose-Einstein condensation takes place at very low temperatures, near the absolute temperature minimum of 273 degrees below zero on the Celsius scale. An exception to this rule could be the behavior of excitons in a crystal. Excitons are combinations of negatively charged electrons and so-called holes - the absence of an electron somewhere in the crystal, leading to a local surplus of positive charge. Pairs of electrons and holes can be bound together and behave like a single bosonic particle, the exciton.
It was predicted in the 1960s that just like other bosons, excitons can form Bose-Einstein condensates. Moreover, this should happen at much higher temperatures than for most other particles – in theory it could happen even at room temperature. Since higher temperatures are much easier to reach in a laboratory setting, excitons could provide an accessible setting in which both the unusual quantum properties of the Bose-Einstein condensates themselves, as well as the unique material properties they bestow upon their host crystals, can be investigated.
M-EELS
Despite the relatively high temperature at which the effect described in the Science article occurs (only 100 degrees centigrade or so below room temperature), and despite the presence of excitons having been suspected for many years, proving beyond doubt that excitons really do form a Bose-Einstein condensate turned out to be surprisingly difficult. The main reason is that there is a different physical phenomenon which is hard to distinguish from a Bose-Einstein condensate of excitons: the formation of a so-called Peierls state, where electrons inside a crystal structure spontaneously organize in a wave-like manner, with alternating peaks and troughs of electron density. Such a wave has many of the same physical characteristics expected for a Bose-Einstein condensate of excitons.
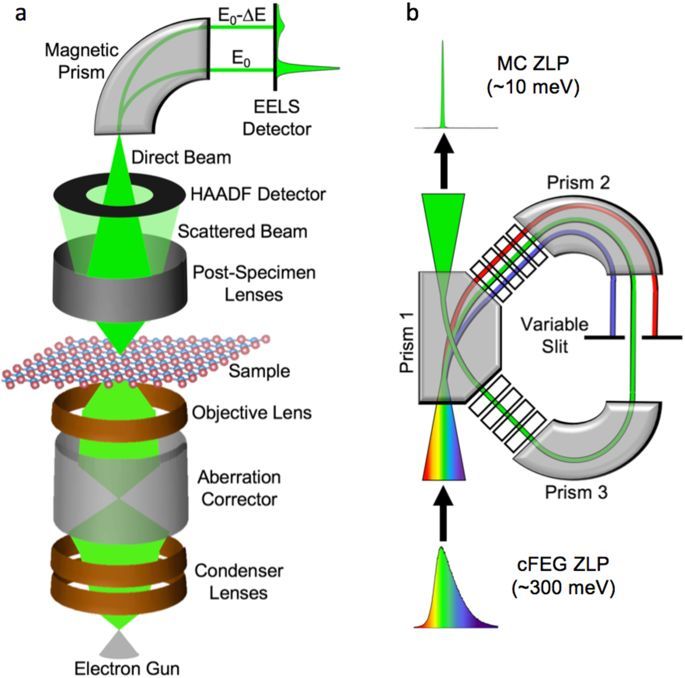
This experiment has now shown that the newly developed experimental technique of Momentum-resolved Electron Energy-loss Spectroscopy (M-EELS for short) does allow them to distinguish unique signatures of condensed excitons in a material called titanium diselenide. This technique developed at the University of Illinois in Urbana-Champaign, and for the first time allows researchers to measure low-energy bosonic particles made of electrons and holes, regardless of their momentum. With this unique capability, the researchers were able to prove that excitons in titanium diselenide spontaneously agglomerate into a Bose-Einstein condensate when the material is cooled down to below 100 degrees centigrade below room temperature.
These measurements for the first time give compelling evidence for the fact that excitons can form a Bose-Einstein condensate at relatively high, easily accessible temperatures. Moreover, they show that M-EELS is a powerful and versatile new technique with many potential future applications.
Signatures of exciton condensation in a transition metal dichalcogenide


REFERENCES
University of Illinois. Available in: https://physics.illinois.edu/news/article/24114. Access in: 29/10/2018.
University of Amsterdam. Available in: https://phys.org/news/2017-12-spontaneous-bose-einstein-condensation-excitons.html. Access in: 29/10/2018.
University of Amsterdam. Available in: https://phys.org/news/2017-12-physicists-discovery-excitonium.html. Access in: 29/10/2018.
0 comments
Sign in or create a free account